The Edge: The Oxyhemoglobin Dissociation Curve, Part 1
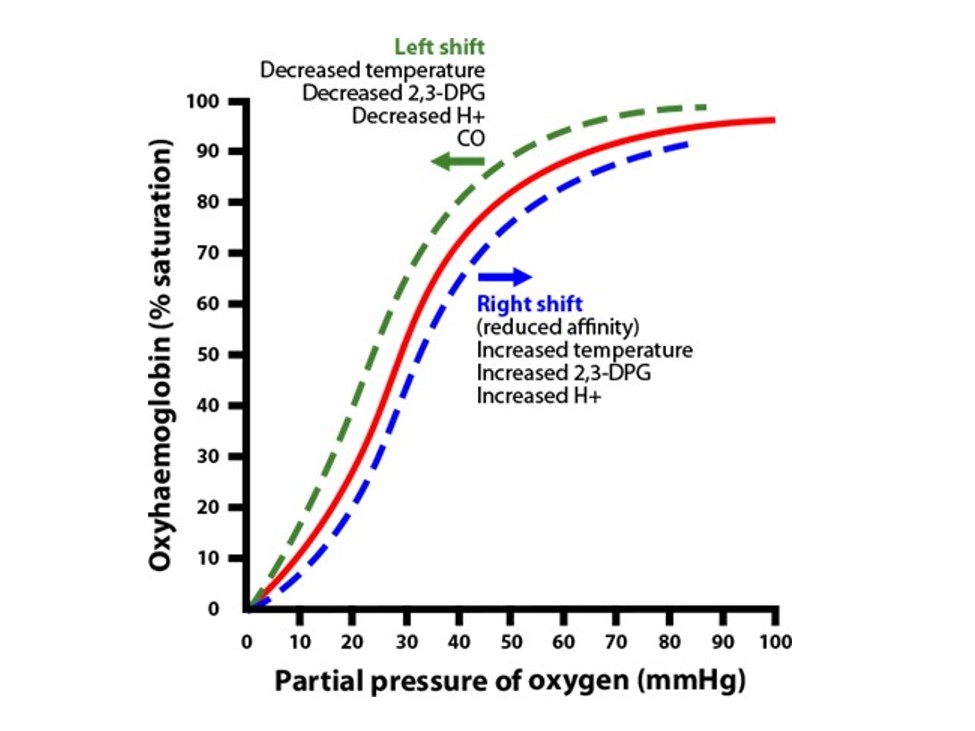
The Edge is a monthly blog series developed by EMS World and FlightBridgeED that features top EMS medical directors exploring the intricacies of critical care in EMS practice. In this installment FlightBridgeED President Eric Bauer, MBA, FP-C, CCP-C, C-NPT, EMT-P, begins a two-part discussion of the oxyhemoglobin dissociation curve.
When we start our careers in prehospital emergency medicine, we may receive different levels of education based on our overall discipline and career goals. We may be taught to a level that misses key physiology that doesn’t fit in the standard paramedic curriculum. One area I found almost mind-blowing was the physiology of the oxyhemoglobin dissociation curve.
Imagine two patients in a hospital room. You are told to treat the more unstable patient. They sit in semi-Fowler’s positions in opposing beds; both appear to be alert and oriented, awake and responding to questions without difficulty. Neither is being treated with oxygen. Patient A has vital signs of BP 126/65, HR 87, and SpO2 88%. In contrast, patient B’s vitals are BP 136/72, HR 93, and SpO2 96%. As you can see, the patients are almost identical. The only difference is their SpO2 readings. So which patient do you treat first?
Common teaching tells us the patient with an SpO2 of 88% is hypoxic and needs oxygen therapy. Is this accurate? Do we put too much weight into SpO2 readings? While it is not wrong to treat the patient with the SpO2 of 88%, it is wrong to automatically think the patient with the SpO2 of 96% is stable and not hypoxic. This is where an understanding of the oxyhemoglobin dissociation curve is essential.
SaO2 and PaO2
The oxyhemoglobin dissociation curve reflects the relationship between the oxygen saturation of hemoglobin (SaO2) and the partial pressure of arterial oxygen (PaO2). It can shift (Figure 1) depending on various factors.
In normal physiology the body needs to offload oxygen to the tissues and muscles and, in contrast, also pick up oxygen within the lungs that is stored on our hemoglobin (Hgb) for future tissue oxygenation. The relative position of the curve represents where in that process the body is. A curve that’s shifted to the right means the Hgb has a lower affinity for oxygen and will thus release its stores of oxygen to the tissues, but also a higher affinity to now allow CO2 and hydrogen ions to bind. With this rightward shift the body can deliver up to 68% of the oxygen stored on the Hgb to the tissues when tissue hypoxia is present (in normal physiology think of exercise).
In contrast, a shift to the left reflects Hgb’s high affinity for bound oxygen and that it won’t release oxygen stores but instead release more CO2 and H+. In normal physiology this is how oxygen is picked up via the lungs and stored on the Hgb for future oxygenation needs. In contrast to the rightward shift, with leftward shifts only 8% of oxygen is released.
If we look at the reasons behind how the body delivers oxygen to the tissues, we can discern the different triggers. A right shift is described by the term Bohr effect.
Bohr Effect
Hemoglobin’s oxygen-binding affinity is directly proportional to the acidity and concentration of carbon dioxide. By using the core reasons behind the low affinity between Hgb and oxygen as a memory tool—think R for raised with a rightward shift, as in raised temp, raised acid, raised 2,3-DPG, and raised PaO2—we can highlight how these points guide our ability to deliver oxygen.
First, raised temperature relates to how our muscles have a high heat potential and account for a large portion of our body heat. Second, raised acid refers to the high concentration of CO2 inside our muscles and tissues based on the oxygenation happening at the tissue level and its byproduct—consider the muscles and tissues a CO2 factory of sorts. This is where our carbonic acid buffering system works behind the scenes in metabolizing the CO2.
Third, raised 2,3-DPG refers to the high concentration of red blood cells within our normal blood content. To put the power of Hgb in perspective, each red blood cell contains 250 million Hgb. Each Hgb can bind with 4 oxygen molecules, creating a potential billion bound oxygen molecules for every red blood cell. As our cells recognize tissue hypoxia, they start releasing excess 2,3-DPG, which is a carbon sugar molecule. The 2,3-DPG enters the red blood cell and binds with any deoxyhemoglobin (Hgb without oxygen bound to it). Once bound it causes a low-affinity state and decreases the hemoglobin’s ability to have bound oxygen. This causes oxygen to instead move freely to the tissues. The 2,3-DPG facilitates this process. The raised PaO2is the final outcome—with the right-shift physiology, the body can deliver oxygen to tissues and muscles efficiently.
The Left Shift
A leftward shift represents the opposite effect. This means the Hgb has a high affinity for bound oxygen and poorly releases bound oxygen stores. In normal physiology this is how oxygen is picked up via the lungs and stored on the Hgb for future needs.
To understand this we must understand the term cooperativity. This means that as the Hgb starts having the first oxygen molecule bind within each seat, it becomes easier for the second, third, and fourth molecules to bind as well. This also reduces the ability of CO2 and H+ to stay bound to Hgb. This is essential to our buffering of H+ and CO2, as it allows them to be released into the bloodstream, where they can be bound with HCO-3 or H2O in the carbonic and respiratory buffering systems. This is known as the Haldane effect.
There’s also a memory tool here: L for low with a leftward shift—as in low temperature, low acid, low 2,3-DPG, and low PaO2. First, low temperature refers to the lungs being exposed to cool, humidified air as we inhale normal tidal volumes. Second, low acid refers to our lungs being more alkalotic. This is due to their ability to get rid of acid efficiently via the respiratory buffer system. Low 2,3-DPG refers to the lungs needing to pick up oxygen. Remember that 2,3-DPG that enters the red blood cells binds to deoxyhemoglobin, causing a state of low affinity. In the lungs the purpose is for oxygen pickup. This means the cells don’t release 2,3-DPG, causing a low 2,3-DPG state. This causes a high-affinity state within the red blood cells, and Hgb is then able to become highly bound with oxygen.
This all happens because the deoxygenated blood returning to the lungs for oxygenation has a partial pressure of oxygen of approximately 40 mmHg. The oxygen entering our oropharynx from the environment has a PaO2 of around 100 mmHg. That is the high concentration. The deoxygenated blood is at approximately 40 mmHg, the low concentration. This sets us up for simple diffusion (Graham’s law). The left shift on the oxyhemoglobin curve reflects this process.
Look for the conclusion of this column in our next issue.
Eric Bauer, MBA, FP-C, CCP-C, C-NPT, EMT-P, is the president and CEO of FlightBridgeED, LLC. He has worked in the EMS field for 30 years, spending the past 19 in the HEMS industry. Eric is an internationally recognized best-selling author, speaker, and educator and holds a Bachelor of Business in Health Care Administration and a Master of Business Administration. He was the recipient of the 2018 John Jordan Award for Excellence in Transport Medicine Journalism by the Air & Surface Transport Nurses Association.